“Trunk disease” is a catch-all term that includes several different fungal diseases of grapevines trunks worldwide. The term was coined in the late 1990s by Dr Luigi Chiarappa 1, and can include foliar and vascular symptoms caused by Petri disease, Black foot, Eutypa, Botryosphaeria dieback, Phomopsis dieback and Esca. Most of the fungi (more than 50 species) causing trunk disease are related, belonging to the order Botryosphareales, but fungi that belongs to the families that forms mushrooms can be also recovered from the cankers. In most of the cases, the cankers interfere with the movement of water from the roots to the leaves, shots and clusters.
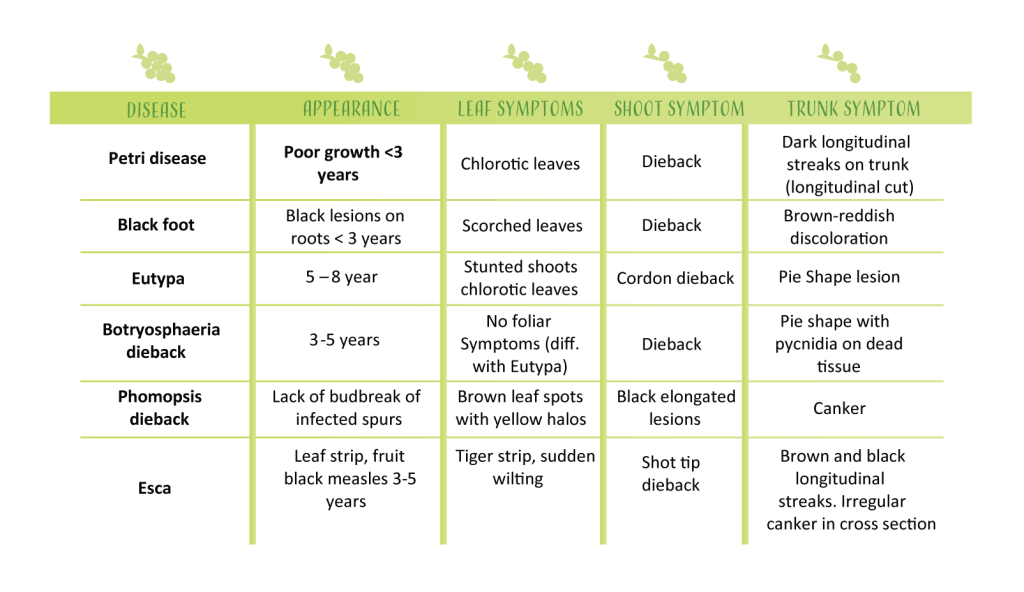
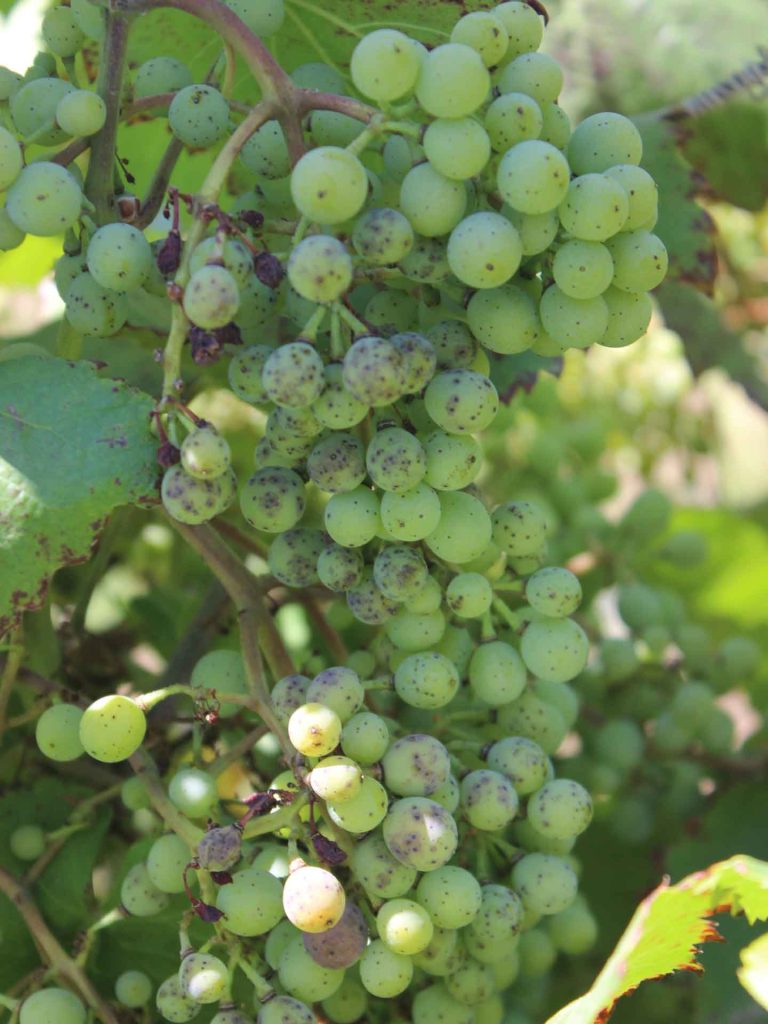
Petri disease and Esca are caused by the closely related species of fungi in the genus Phaeomoniella and Paheoacremonium. In both diseases, longitudinal black stripes are visible under the bark. Different from Petri disease which occurs in vines younger than three years, Esca is observed in more mature plants. The foliar symptom can include the well-known “Tiger Stripe” pattern (photo 1). This symptom is visible normally by the end of June, when high temperatures stress the vines. Reddish-brown patches on the leaves are observed in red cultivars, while yellow patches are more common on white grapes. Esca is also known as “black measles” because the dark spots that develop on berries (photo 2). Measles is particularly damaging to table grapes because berry appearance is paramount.
Black foot is caused by two species of the fungal genus Cylindrocarpon, and it most commonly observed on young vines (three-year-old vines or younger). Stunted vines and scorched leaves (photo 3) are a typical sign of black foot. Affected roots present black lesions and look like they are dead. The disease was reported by late 1990’s in California and has been frequently found in fields with poor planting practices, especially those that were J-rooted at planting. The disease if not prevented, can require costly replanting.
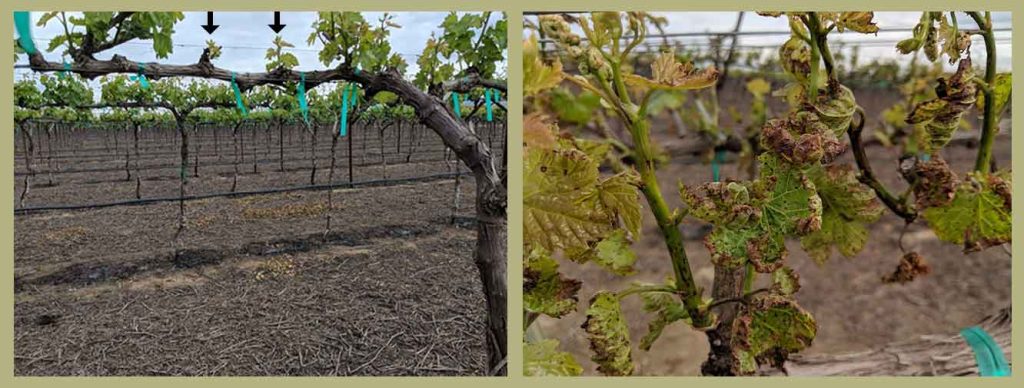
Botryosphaeria is caused by more than 20 species of fungi. However, fungi in of the species Lasiodiplodia and Neofusicocum are the most damaging. Spurs from infected vines won’t develop new shoots (Photo 4) . In cross-section, a pie shape necrotic lesion can be observed in infected trunks (Photo 5), however this symptom can be also observed in vines infected with Eutypa.
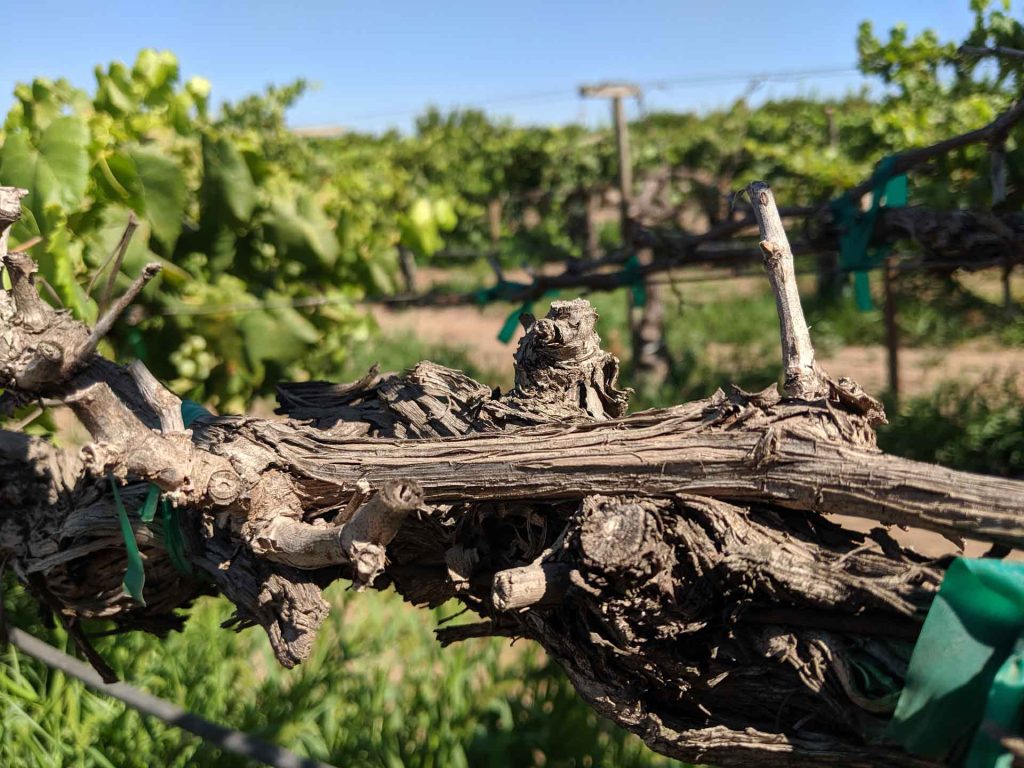
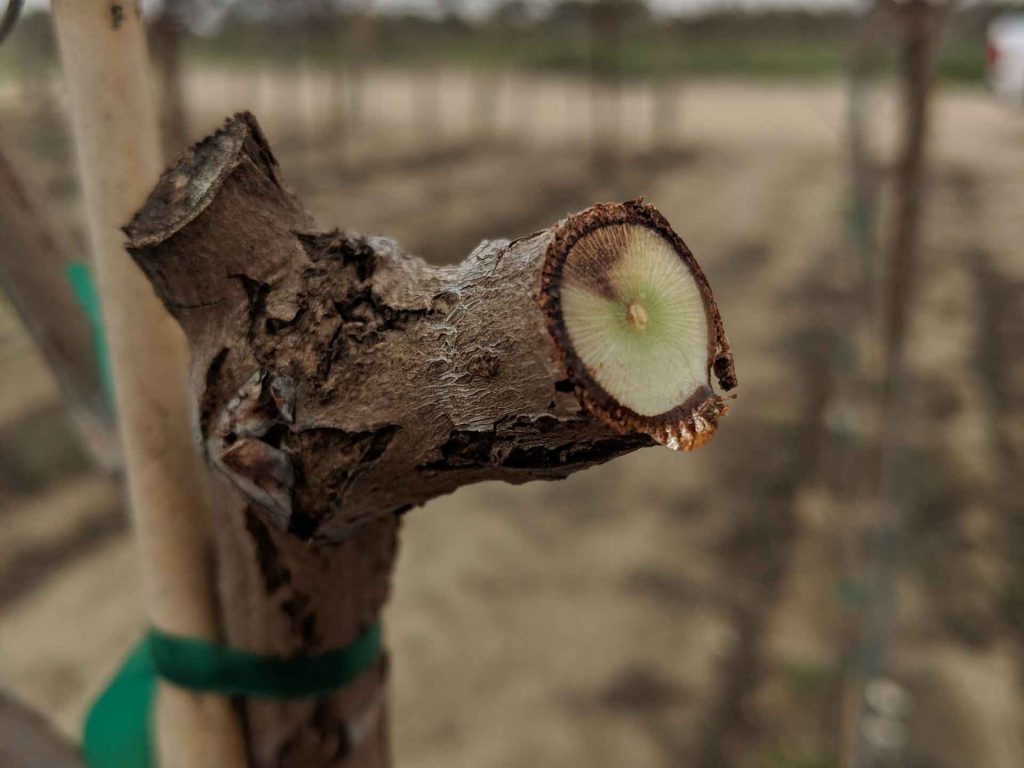
Eutypa is a common disease in California, and it is caused by the fungus Eutypa lata. In addition to the pie shaped internal lesion, proliferation of stunted shoots is common (Photo 6). This symptom is absent in Botryosphaeria disease, where no growth or no leaf symptoms are observed.
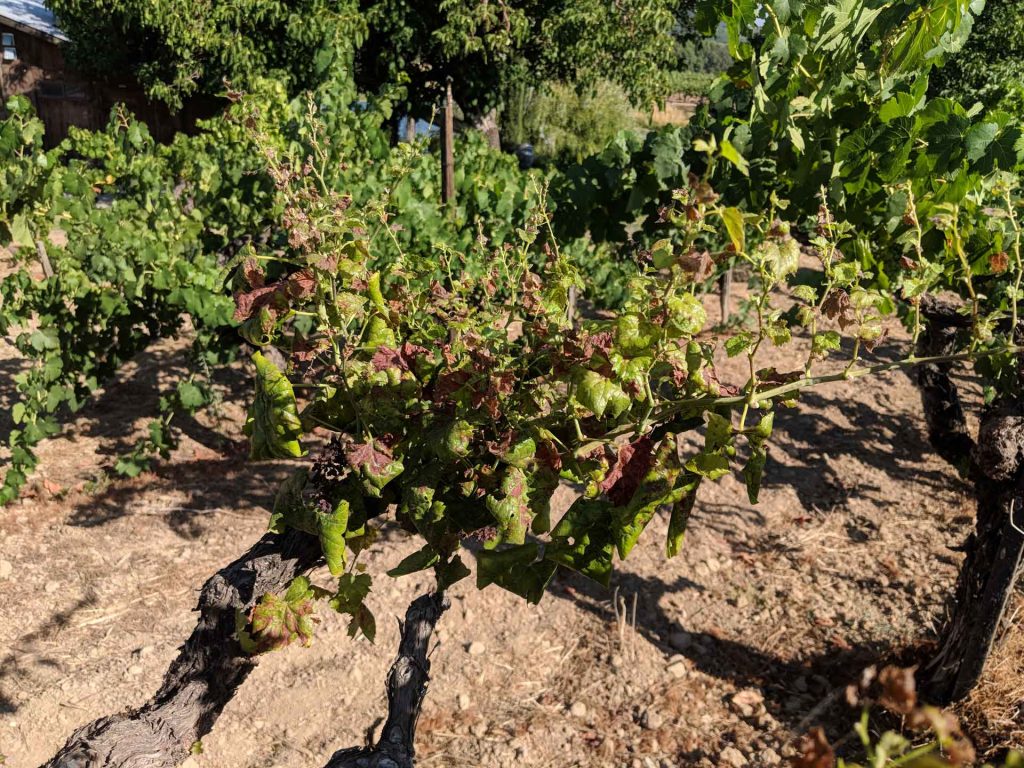
Phomopsis is normally associated with damage on green tissue, specially canes and leaves. However, when conditions favor the pathogen, damage caused by Phomopsis can result in dead of spurs, canes and buds. Severely affected canes develop cracks and have a bleached appearance during winter. In early spring reproductive structures of the pathogen are visible as black speckles.
Impact
All the described trunk diseases can be seen at any time of the vineyard life, but normally they start to appear between the third and the fifth year after planting. They can appear alone, or in combination, which can exacerbate the plant stress. In general, by year 10 to 12, 20 percent of the plants show symptoms when no preventive actions have been implemented. At this point, trunk diseases enter in an exponential phase, reaching 75 percent of infection by year 15, and 100 percent by year 20.
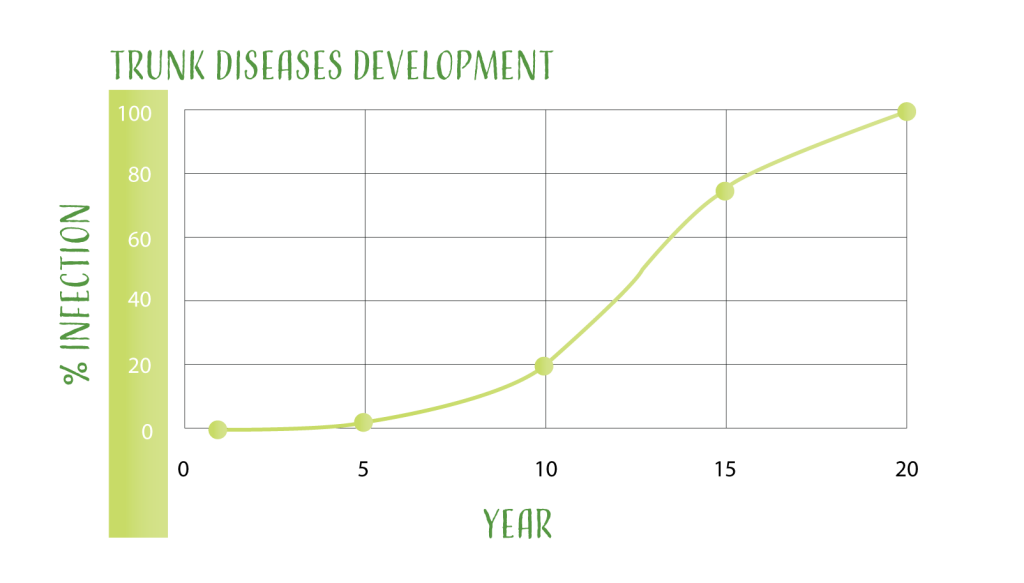
Significant losses are associated with trunk disease development. Reduction in number of clusters, decrease in quality and cosmetic damages are the most visible impacts in the field. However, cost of replanting, and/or retraining if grower elects to use vine surgery, especially in young vineyards, it also a major cost associated with trunk diseases. Siebert2 in 2000, estimated the annual loss caused by Botryosphaeria and Eutypa in the California wine grape industry was $260 million. Similar negative effects have been reported in other grape growing areas and trunk diseases.
Management
Trunk diseases are considered chronic diseases, and unfortunately there is no fungicide that can provide curative action. Preventive management or the removal of infected tissue from the vine (surgery) and destruction by mulching or soil incorporation of infected shoots and canes are the only viable alternatives.
More than 95 percent of trunk diseases infections are associated with pruning, or other cultural practices that leave pruning wounds exposed at a time when the wounds may be infected. Dispersal of the pathogens responsible for causing trunk disease occurs during rain events. Under California conditions pruning and rain overlap during the winter months (November-January). Dr. Gubler and his team found that delaying pruning closer to bud break significantly reduces disease3. The logic behind this is that in a typical California year, rain is gone by the end of February and the days are warmer, letting the plants recover sooner than during the colder days of December and January. In addition, some sap movement (bleeding) starts to be present in February, helping the plant to remove the infective spores from susceptible tissue.
However, and knowing that the labor and logistics doesn’t permit all growers to postpone pruning until the last part of the winter, the use of fungicides to protect the exposed tissues is important to reduce the rate of the infection. The best practice is to protect the plant any time there are pruning wounds. This is especially important if rain is expected following pruning. If pruning is done in November or December, it is advised at least two sprays with protectant fungicides be applied. If the pruning is postponed until January and warmer days are forecasted, one protectant spray after pruning is ideal.
Another strategy for pruning is to do a double pruning (pre-pruning + pruning). It consists of pre-pruning the vines between November and January. Then, by the end of February or March, the pruning process is completed. The objective of this method is to remove any potential infection occurred during the winter months. A complementary fungicide spray after the last pruning can increase the control of trunk diseases.
In order to improve the efficacy of protective fungicide, a closer identification of the disease is recommended as any particular fungicide cannot control all possible pathogens. A recent report done by Baumgartner and Brown4 on their research in 2017, demonstrates that Pristine, Topsin + Rally (in mixture), and Luna had better preventive control of Botryosphaeria and Phomopsis dieback. Eutypa was controlled more effectively with Pristine. The highest control of esca was obtained with Serifel, but it only reached 64 percent. Results in 2018 in the same study presented different results and were mainly associated with a different weather condition.
Different studies, including the one recently done by Dr. Baumgartner and collaborators5, demonstrates that preventive practices works better if they are established during the first years of the crop (Figure 2). Dr. Baumgartner estimated that when more effective practices are adopted early in the crop life, it is expected to prolong the vineyard rentability by at least 25 years.
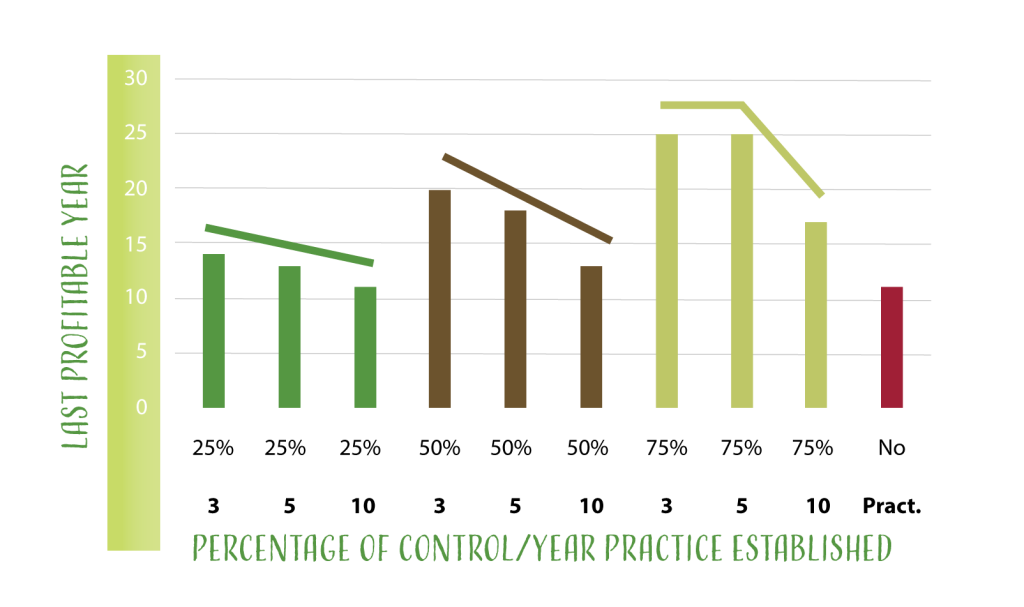
Further information on trunk diseases can be found at:
1) http://ipm.ucanr.edu/PMG/selectnewpest.grapes.html
2) Bettiga LJ, ed. Grape Pest Management, Third Edition. University of California, Agriculture and Natural Resources; 2013. https://books.google.com/books?id=4A9ZAgAAQBAJ.
3) Wilcox WF, Gubler WD, Uyemoto JK, eds. Compendium of Grape Diseases, Disorders, and Pests. Second. St Paul, Minnesota, USA.: The American Phytopathological Society; 2017.
4) Disease P, Gramaje D. Grapevine Trunk Diseases: Symptoms and Fungi Involved. 2018;102(1):12-39. https://apsjournals.apsnet.org/doi/10.1094/PDIS-04-17-0512-FE
Cited literature:
1. Gramaje D, Úrbez-Torres JR, Sosnowski MR. Managing Grapevine Trunk Diseases With Respect to Etiology and Epidemiology: Current Strategies and Future Prospects. Plant Dis. 2018;102(1):12-39. doi:10.1094/PDIS-04-17-0512-FE
2. Siebert JB. Economic Impact of Eutypa on the California Wine Grape Industry. Davis; 2000.
3. Gubler WD, Rolshausen P e., Trouillase FP, et al. Grapevine trunk Diseases in Califronia. Pract Winer Vineyard. January 2005:1-9.
4. Baumgartner K, Brown AA. Protectants for Trunk-disease management in California table grapes. In: California Table Grape Seminar. Visalia: California Table Grape Commission; 2019:19-21.
5. Baumgartner K, Hillis V, Lubell M, Norton M, Kaplan J. Managing Grapevine Trunk Diseases in California ’ s Southern San Joaquin Valley. 2019;3:267-276. doi:10.5344/ajev.2019.18075