Potatoes require high levels of potassium and nitrogen to achieve optimum yields. Potassium (K) is commonly applied as potassium chloride (KCl) while nitrogen (N) is applied both preplant and in season through fertigation. Crop N is managed in season through tissue testing of potato petioles for nitrate. Petiole nitrate levels are then used as a diagnostic tool for in-season N fertilizer applications based on extension recommendations.
Methods
This research was conducted in the Columbia Basin in Hermiston, Ore. Soils in this area are sandy, which increases leaching potential for nitrate and other anions, like chloride (Cl). Previous research found that petiole nitrate levels decreased as petiole Cl increased. There was concern that there was an antagonism in uptake of nitrate and Cl and that if in-season fertilizer recommendations based on petiole nitrate levels did not consider petiole Cl, growers might be applying more N than was required for crop demand. In other words, there was a concern that petiole nitrate levels might be lower not because of low soil N, but rather because of high Cl uptake following KCl application. This project was designed to understand Cl dynamics in potato production systems.
In this project, source and timing were included as treatments while rate and placement were consistent for all treatments. Three K sources (potassium chloride (KCl); sulfate of potash, K2SO4 (SOP); and K2SO4*2MgSO4 (KMag)) were applied at 200 lb K per acre at three different times in the season. The timing of the treatment applications were 206 days prior to planting (fall preplant), 14 days prior to planting (spring preplant) and 35 days after planting (layby). Russet Burbank potatoes were planted on April 11. After planting, beds were tilled by moving soil from between the rows into the potato rows. Potato plants had emerged but not closed canopy at the time of the layby fertilizer application.
Potato petioles were collected two times in the growing season (70 and 97 days after planting) to coincide with extension recommendations for in-season fertilizer management decisions. Soil samples were collected 68 to 70 days after planting. Aboveground whole plant biomass was collected 116 days after planting and potato tubers were harvested 21 days later. Potato yield and quality metrics including specific gravity were evaluated for all treatments. These plant and soil measurements were designed to understand how much Cl plants were taking up, where in the plant the Cl was going and how Cl uptake was impacting plant N levels.
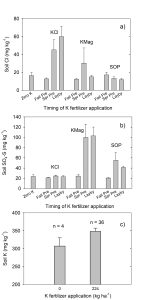
Source by Timing Interaction Revealed
Soil Cl concentrations revealed a source by timing interaction. When KCl was applied in the fall, soil Cl levels were similar to those in zero K control as well as KMag and SOP treatments; however, when KCl was applied in spring or during the growing season, soil Cl was greater with KCl compared to other treatments. Plant Cl measurements followed the same pattern as soil measurements. The most likely explanation for these results was greater leaching of Cl below rooting depth with fall fertilizer application as compared to spring or in-season fertilizer applications.
Despite being in a low-rainfall area, several factors favored overwinter leaching of Cl below the root zone. First, the soil texture is sandy loam with a low water-holding capacity. Second, the timing of field operations and bed preparation relative to fall fertilizer application facilitated Cl exclusion from beds. In the fall, fertilizers were applied to flat ground. Prior to potato planting in spring, beds were created from the top 2 to 4 inches of the soil present on flat ground. Therefore, overwinter leaching of Cl below a depth of approximately 4 inches would be sufficient to move it below the potato beds. Cumulative rainfall between fall preplant fertilizer application and planting in spring was 4.25 inches, though daily precipitation was never more than 0.4 inches. From February 1 to March 18 (date of spring preplant fertilizer application), cumulative rainfall was 2 inches as compared to cumulative estimated ET of 0.83 inch, which indicates a potential for leaching. In contrast, during the interval between spring preplant fertilizer application and planting (March 28 to April 11), cumulative rainfall was 0.3 inches as compared to cumulative estimated evapotranspiration (ET) of 0.43 inches. Therefore, little or no leaching was expected between spring preplant fertilizer application and planting. Following potato planting, irrigation plus precipitation did not leach out Cl.
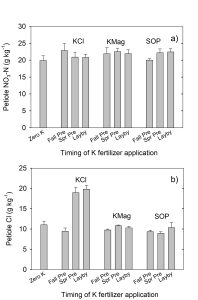
Petiole nitrate concentrations were similar for all treatments at both sampling dates. Higher Cl levels in petioles with spring preplant or layby KCl application did not reduce petiole nitrate. For example, at 70 days after planting, petiole Cl was increased twofold with spring preplant or layby KCl application as compared with fall application. In contrast, petiole nitrate concentrations were similar regardless of the timing of KCl application. At harvest, crop N concentrations were similar across all treatments.
K fertilizer source and timing did not impact total or marketable potato yield. There were no significant differences for specific gravity by treatment. However, among KCl treatments, the values for specific gravity and potato yield were as follows: Fall Preplant > Spring Preplant > Layby. This suggests that higher soil and plant Cl during the growing season may have delayed tuber initiation or growth. Typically, specific gravity increases with tuber maturity.
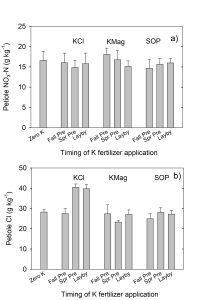
This experiment was designed to minimize yield differences between treatments so that nutrient movement could be evaluated without regard to plant nutrient partitioning and physiological source-sink relationships. These results indicate potato plants accumulate large concentrations of Cl when available due to KCL being applied later in the growing season. Fall KCl applications, which allowed for overwinter leaching below the root zone, resulted in the lowest soil and plant Cl concentrations when compared to other application times.
In this research, aboveground biomass and tubers were collected three weeks apart and the Cl concentration in aboveground biomass was higher than in tubers. Peak nutrient uptake in potatoes occurs during times of significant aboveground growth. Photosynthates are then translocated into potato tubers during tuber bulking, which subsequently increases water uptake into tubers. As a result, Cl concentration in the tubers is diluted and decreases as a proportion of tuber weight during this bulking stage. Standard grower practice is to leave desiccated vines in the field after harvest. The majority of plant Cl is in the aboveground biomass at the end of the season and thus Cl is added back into the soil.
In this project, we did not measure an antagonism in crop update between nitrate and Cl. Our experimental design likely minimized the interaction between N and Cl because N was applied at lower rates during peak uptake to meet crop demand. Total N concentration in aboveground biomass and potato tubers as well as nitrate in petioles were generally unaffected by K source or time of K application. In this research, petiole Cl levels were affected by K source and time of application. Concentrations increased when KCl was the K source, and as KCl was applied closer to petiole sampling date. In this project, Cl levels were always higher in petioles collected later in the year, which indicates potatoes continue to accumulate Cl throughout the growing season. Petiole nitrate levels, by contrast, were consistently lower among all treatments for the second petiole collection date. Although Cl is an essential micronutrient, it is not metabolized into plant compounds, and high concentrations of Cl are maintained in aboveground plant tissue including petioles throughout the growing season.
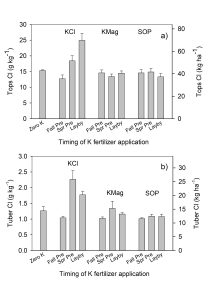
Though there were significant differences in petiole Cl concentrations by treatment, the antagonism in uptake between N and Cl in petioles that had been documented by other researchers was not measured in this study. In this project, N was applied weekly at a uniform rate to all treatments during periods of peak uptake throughout the growing season and was thus replenished and available for plant uptake. This application method likely allowed the Cl uptake and movement to be unaffected by N availability.
Potato plants can take up Cl when it is available, and that Cl accumulates in plant tissue (particularly aboveground biomass) until harvest. Higher concentrations of petiole Cl from preplant or in-season KCl application did not affect petiole nitrate when N was applied via fertigation throughout the growing season. Fall-applied Cl was not taken up by the crop because of the opportunity to leach out of the soil used to form potato beds prior to planting. Even in a low-rainfall area, sufficient leaching of Cl below the rootzone is possible if KCl is applied far in advance of planting.
This project was funded by United States Department of Agriculture: National Institute of Food and Agriculture and Compass Minerals. Dan Sullivan worked on data analysis and publication of results. Dr. Don A. Horneck proposed this research project and died before the completion of this project. He is missed.
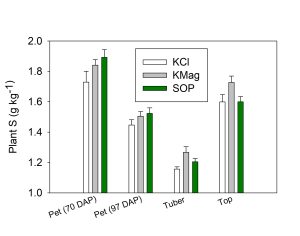