Growers and PCAs should be on the lookout for a new pest called carpophilus beetle (Nitidulidae: Carpophilus truncatus) (Figures 1 and 2).
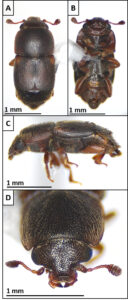
Damage occurs when adults and larvae of this pest feed directly on the developing kernel, causing reductions in both crop yield and quality. This pest was initially found infesting almonds and pistachios in the northern and central part of the San Joaquin Valley, and a broader survey is now underway to verify the extent of its spread in California.
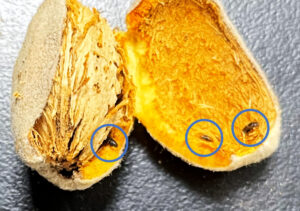
The carpophilus beetle is recognized as one of the top two pests of almond production in Australia, where growers typically experience 2% to 5% infestation, but it can be closer to 30% to 40% in more extreme cases (Madge 2022). In addition to feeding on new crop nuts, the carpophilus beetle can also likely facilitate the spread of Aspergillus fungi that can lead to the production of aflatoxins, which are known human carcinogens that are heavily regulated in key markets.
Biological and chemical control options are very limited or unknown. As such, the key to managing carpophilus beetle is crop sanitation since this pest overwinters on remnant mummy nuts in the orchard like navel orangeworm (Pyralidae: Amyelois transitella) (NOW). Growers and PCAs can monitor carpophilus beetle by directly inspecting mummy or new crop nuts, although there are no known economic thresholds for this pest.
Global Distribution and Initial Detections in California
Carpophilus beetle has been well-established in Australia for over 10 years, where it is considered a key pest of almonds. More recently, the beetle was reported from walnuts in Argentina and Italy. Carpophilus truncatus is a close relative to other beetles in the genus Carpophilus, such as the driedfruit beetle (C. hemipterus) that is known primarily as a postharvest pest of figs and raisins in California.
In California, populations of carpophilus beetle were first detected in September 2023 in almond and pistachio orchards in Madera and Kings counties, respectively. Pest identification was subsequently confirmed by CDFA. A broader survey of orchards throughout the San Joaquin Valley is now underway to determine the extent of the outbreak as well as confirm additional hosts such as walnuts and pecans. So far, almond or pistachio orchards infested by carpophilus beetle have been confirmed in Stanislaus, Merced, Madera and Kings counties, suggesting the establishment of this new pest is already widespread. In fact, some specimens from Merced County were from collections that were made in 2022, suggesting the pest has been present in the San Joaquin Valley for at least a year already.
Host Plants
While in Australia the carpophilus beetle is primarily found in and around almond orchards, alternative host plants have been identified that the beetle can use for feeding and reproduction. These include Brazil nuts (Lecythidaceae: Bertholletia excelsa), candlenuts (Euphorbiaceae: Aleurites moluccanus), cashews (Anacardiaceae: Anacardium occidentale), pistachios (Anacardiaceae: Pistacia vera), quandong seeds (Santalaceae: Santalum acuminatum), walnuts (Juglandaceae: Juglans regia), acacia seeds (Fabaceae: Acadia spp.), pine nuts (Pinaceae : Pinus spp.), desiccated coconut flesh (Arecaceae: Cocos nucifera), sunflower seeds (Asteraceae: Helianthus annuus) and granulated or powdered pollen (Madge 2022). While adults have also been shown to survive on dried apricots (Rosaceae: Prunus armeniaca), dates (Arecaceae: Phoenix dactylifera) and cup mushrooms (Pezizaceae: Peziza spp.), the larvae are unable to complete development on these hosts (Madge 2022). In California, this pest has so far been confirmed feeding on almonds, pistachios and walnuts.
Seasonal Ecology
Carpophilus beetles overwinter in remnant nuts (i.e., mummy nuts) that are left in the tree or on the ground following the previous year’s harvest. In contrast to NOW, carpophilus beetles tend to prefer remnant nuts on the ground, likely due to increased moisture, and they generally tend to forage closer to ground level (Madge 2022). That said, carpophilus beetles can also be found in tree mummies as well.
The beetles become active when temperatures and photoperiod increase in the spring. They can have multiple generations per year, although there is no information available on the upper and lower temperature thresholds for this pest, much less degree-day requirements. While more research is still needed to better characterize beetle activity in the spring prior to hull split, they likely make use of mummy nuts as a reproductive host during that early part of the season, similar to NOW.
At hull split, carpophilus beetles will move onto new crop nuts, although some fraction of the population does remain on mummies all year. Prior to egg deposition on new crop nuts, the adult beetles will chew through the shell (Figure 3) and/or feed on the kernel, which is thought to both facilitate larvae access to the developing kernel, as well as inoculate the nut with a symbiotic gut-associated yeast that likely help the larvae feed (Madge 2022). Recent studies in Australia have demonstrated adults prefer to oviposit onto nuts previously fed upon by other carpophilus beetles and/or inoculated with yeasts (Madge 2022). While carpophilus beetles have been found infesting nuts throughout the tree, they tend to concentrate in the lower canopy (Madge 2022). This contrasts with NOW, which tend to initially infest nuts higher in the tree canopy.
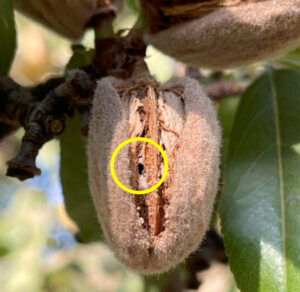
Following egg deposition, the larvae that emerge feed on the developing kernels, leaving the almond kernel packed with a fine powdery mix of nutmeat and frass that is sometimes accompanied by an oval-shaped tunnel (Figures 4 and 5).
- Figure 5. Carpophilus truncatus feed directly on the nut kernel, which can sometimes result in a distinct oval-shaped tunnel (photo by H. Wilson.)
Damage from carpophilus beetle may be confused with NOW and/or ants, but these can be differentiated. For example, NOW tends to feed all over the kernel (rather than tunneling) and produces a darker and larger type of frass (i.e., excrement) along with webbing (Figure 6).
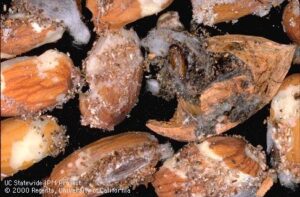
In contrast, ants tend to chew through the skin of the kernel and feed primarily on the white nutmeat, leaving the papery skin behind (Figure 7).
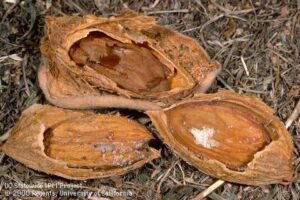
Ant feeding is also associated with the presence of a fine white powder (similar to carpophilus beetle damage) that can be seen while sampling in the field but disappears in the hulling/shelling process prior to the inspection of processed kernels (Figure 8).
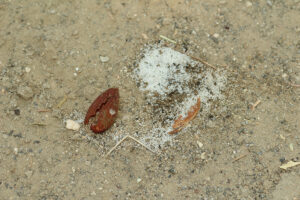
Monitoring
Monitoring for carpophilus beetle is currently limited to direct inspection of hull-split nuts for the presence of feeding holes and/or larvae or adult beetles. Given their tendency to infest nuts lower in the canopy, this is a good area to focus on for initial inspection of new crop nuts. That said, to get an idea of average overall infestation levels, it is best to take a sample from freshly shaken new crop nuts on the ground at harvest, since this is more representative of the entire tree canopy.
Over the winter, mummy nuts can also be inspected for the presence of carpophilus beetles. Initial studies from Australia suggest that monitoring points should be at least 200 yards apart (Madge 2022). While no specific economic thresholds have been developed, summer infestations on new crop nuts tend to reflect the distribution of infested mummy nuts during the winter.
A lure based on male-produced aggregation pheromone from three related species of Carpophilus spp. was previously developed in Australia and used for monitoring as well as an attract-and-kill strategy in stone fruit orchards (Hossain 2018). With the arrival of C. truncatus in Australia, this monitoring and management strategy was tested in almond orchards. Unfortunately, in its current form, this trap and lure system is not very attractive to C. truncatus, and so it has no utility for either monitoring or as a control strategy (Hossain 2018).
As such, Australian researchers are now working to develop a pheromone lure that is specific to C. truncatus as well as one or more co-attractants based on host plant volatiles and/or gut-associated yeasts. Preliminary studies have shown a lot of promise, and this new lure may soon provide a better monitoring tool for growers, PCAs and researchers, but it is not yet commercially available (Madge 2022). Initial studies with the new species-specific pheromone lure have demonstrated that carpophilus beetles appear to forage mostly at ground level, and beetle catch with the candidate pheromone was improved when traps were moved from the tree canopy (i.e., hung at about 5 ft height) to ground level (Madge 2022).
Biological Control
While there are certainly parasitoids and predators that attack Nitidulids and species in the genus Carpophilus in particular, very little is known about the specific natural enemies of C. truncatus, much less their efficacy in an orchard setting. Much more information exists on the parasitoids of C. hemipterus, the driedfruit beetle, a key pest of figs and stone fruit in California. Key parasitoids of C. hemipterus include the Encyrtids Zeteticontus spp. and Cerchysiella spp. as well as a Proctotrupid Brachyserphus abruptus, all of which attack the larvae. Additional parasitoids include the Braconid Microctonus nitidulidis and the Bethylid Pseudisobrachium flavinervis, which attack the adult and pupal life stages, respectively. These parasitoids have a wide host range and may attack C. truncatus, but more research will be necessary to confirm this as well as their efficacy for population control.
Documented predators of Carpophilus spp. include the Staphylinid Atheta coriaria and the Reduviid Peregrinator biannulipes. Other generalist predators commonly found in tree nut orchards (e.g., spiders, lacewings) may also contribute to biological control of C. truncatus, but again more research is needed to further characterize this, much less determine their impacts on C. truncatus specifically.
In the absence of specialist parasitoids or predators, researchers in Australia have focused on the use of entomopathogenic fungi (EPF) for carpophilus beetle, particularly the EPF Beauvaria bassiana. While preliminary studies found that in some cases B. bassiana could cause up to 70% mortality of C. truncatus larvae (and to a lesser extent adults), the use of this EPF under field conditions is still being developed (Madge 2022).
Finally, vertebrates like birds and rodents may provide some degree of control by consuming or damaging remnant mummy nuts infested by carpophilus beetle, but the impacts of this remain unclear in California orchards.
Chemical Control
The ability to control carpophilus beetle with insecticides is limited, primarily due to challenges with spray coverage. Most of the beetle’s life cycle is spent protected inside the nut, with relatively short windows of opportunity available to spray the adults while they are exposed. In Australia, the use of bifenthrin for control of carpophilus beetle has produced inconsistent results, and experiments to improve coverage with various adjuvants did not lead to improved control (Madge 2022). Furthermore, the continued presence of carpophilus beetles on remnant mummy nuts throughout the season presents an additional challenge to control with insecticides.
Cultural Control
In the absence of clear chemical or biological control strategies, the most important tool for managing carpophilus beetle is crop sanitation. In Australia, this is currently the primary method for managing this pest. While carpophilus beetle can be found overwintering on remnant mummy nuts both in the tree canopy and on the ground, they tend to prefer ground mummies, likely due to elevated moisture conditions. After removing remnant mummy nuts from trees, it is critical all ground mummies be thoroughly broken apart and destroyed. Simply disking mummies under the soil will not be effective, since research in Australia demonstrated even when mummies are buried as deep as 3 ft down, adult carpophilus beetle can still survive and crawl up to the soil surface (Madge 2022). As such, make sure to use a flail mower to thoroughly destroy mummy nuts. It might need a double pass to ensure all nuts are shredded.
Carpophilus beetle is a new pest in California that will need to be addressed by both researchers and growers alike. Within the research community, new research and extension activities are being developed by UCCE personnel in collaboration with their counterparts in Australia. Until more is known about this pest in California, growers are advised to monitor for its presence and follow the Australian model of focusing on winter sanitation as the primary means for its control.
If you suspect that you have this beetle in your orchard, please contact your local UCCE Farm Advisor (https://ucanr.edu/About/Locations/), County Agricultural Commissioner (https://cacasa.org/county/) and/or the CDFA Pest Hotline (https://www.cdfa.ca.gov/plant/reportapest/) at 1-800-491-1899.
Selected References
Hossain, M. 2018 “Final Report – Management of Carpohilus Beetle in Almond” Project Code AL15004, Horticulture Innovation Australia, North Sydney, Australia.
https://www.horticulture.com.au/growers/help-your-business-grow/research-reports-publications-fact-sheets-and-more/al15004/
Madge, D. 2022 “Final Report – An Integrated Pest Management program for the Australian almond industry.” Project Code AL16009, Horticulture Innovation Australia, North Sydney, Australia.
https://www.horticulture.com.au/growers/help-your-business-grow/research-reports-publications-fact-sheets-and-more/al16009/