The San Joaquin Valley (SJV) is in the midst of an ongoing drought. Total precipitation in Fresno from October to March was 7.3 inches which amounts to 60% of the historical average of 12 inches. With little or no rain in the forecast and in anticipation of another dry and hot summer, growers might reflect on some environment-related issues observed in 2021.
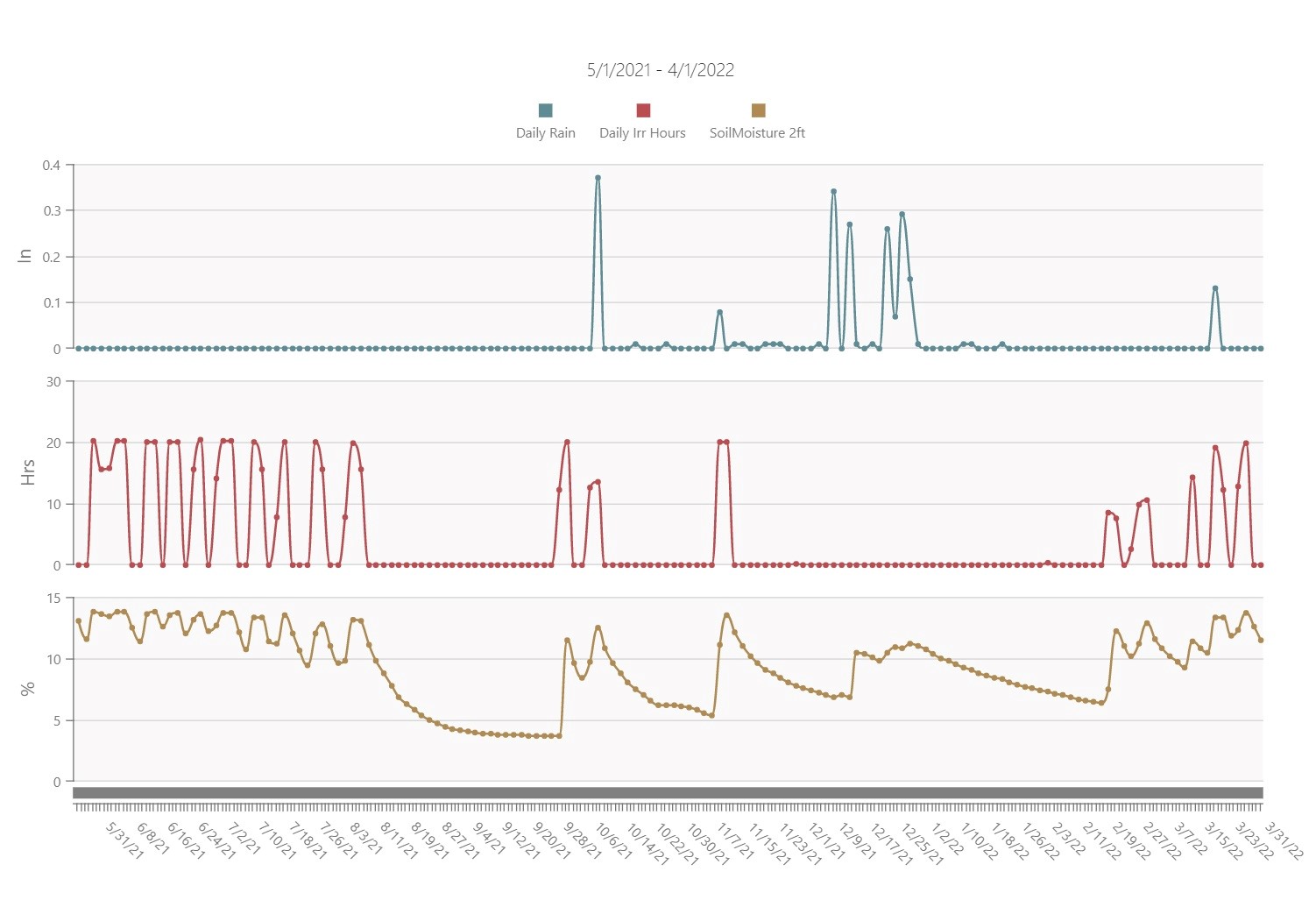
Maintaining Adequate Soil Moisture is Critical
Many growers who suffered greatly from delayed spring growth (DSG) in 2021 allowed the soil to become too dry the preceding fall or winter. Sufficient carbohydrate content of the vines and adequate soil moisture are the keys to dodge DSG. Healthy carbohydrate content in the vines can be attained with a balanced canopy vs yield and a pest-free canopy kept in good condition postharvest. Adequate soil moisture can be achieved through postharvest irrigation or even winter irrigation if precipitation is lacking. Postharvest irrigation helps prevent early defoliation, reduces the chance of winter freeze damage, helps leach any accumulated salts and helps rehydrate the vines after they emerge from dormancy.
Thus, a key lesson learned from 2021 should be the importance of maintaining soil moisture during the winter months. The beginning of last winter saw record precipitation, and many growers took proactive measures to prevent DSG in the dry second half of winter in early 2022. For example, in Figure 1, postharvest irrigation (end of September), winter irrigation (mid-November) and early spring irrigation (mid-February) have been noticed from this vineyard, which is one of the collaborative sites contributing to the UC IPM weather station networks (ipm.ucanr.edu/weather/grape-powdery-mildew-risk-assessment-index). As a pilot study site, we added a pressure switch and soil moisture probes at soil depth of 1, 2 and 3 feet to help growers improve their irrigation scheduling. We are glad to see growers are taking advantage of those data to manage vineyard water to reduce the risk of DSG.
Ideally, a regional soil moisture content network could provide critical winter soil moisture information to guide growers near the station to decide whether to irrigate the vines during the winter month.
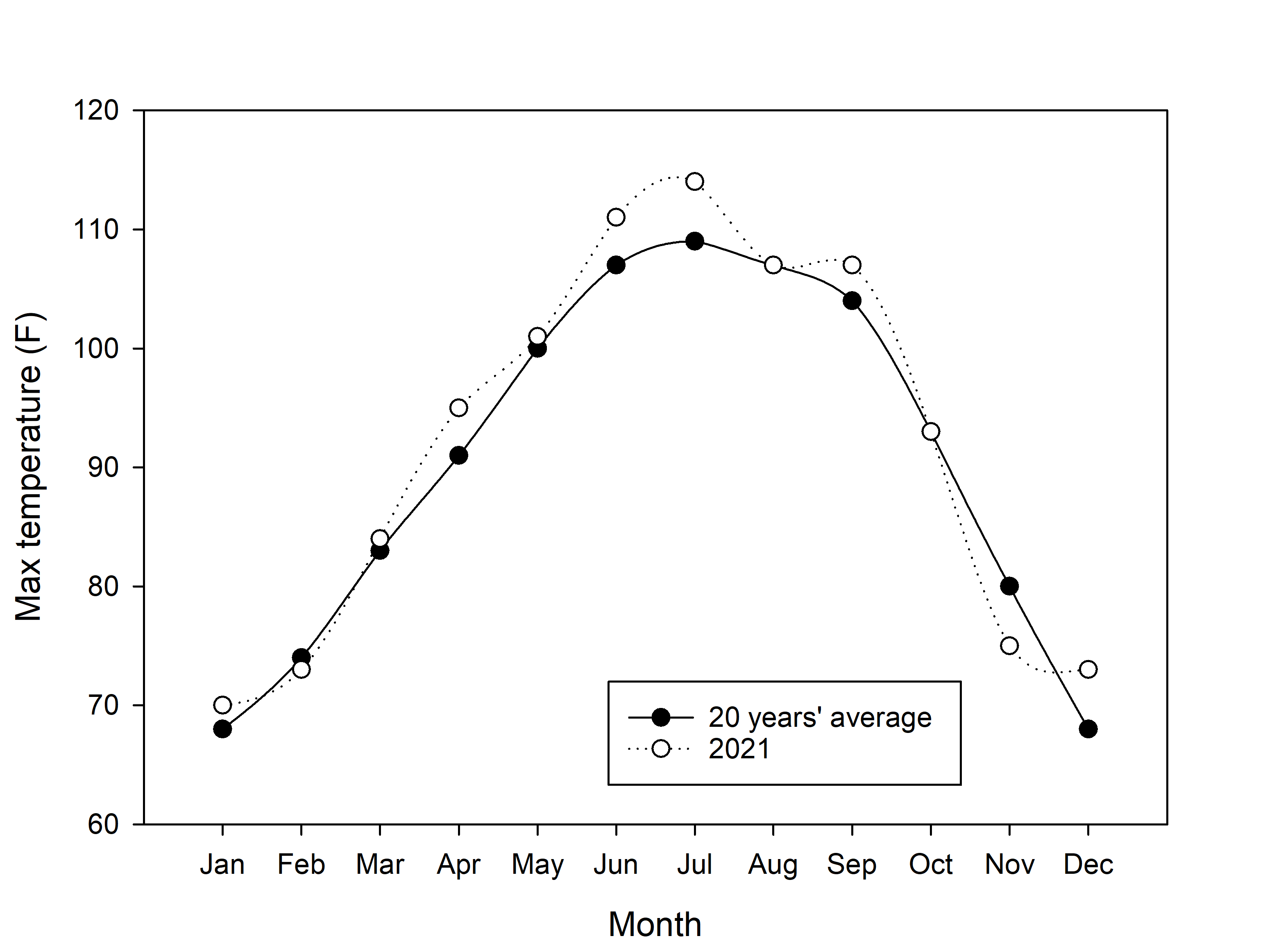
Cultural Practices Can Help Protect Against Heatwaves
Besides the severe winter drought, growers also experienced record summer heat in 2021. Max monthly temperature in 2021 was much higher than last 20 years’ average, especially in June and July (Figure 2), and monthly reference evapotranspiration (ETo) in 2021 was also higher than the last 20 years’ average (Figure 3). Sun-exposed clusters and berries under the extreme summer heat will develop sunburn, which will reduce fruit yield and quality, including Brix and raisin B&B grade. I have written a previous article about grapevine heat stress and sunburn management (Progressive Crop Consultant May/June 2019), and many tools are available for growers:
- When developing a new vineyard, row orientation and trellis design can help minimize direct sun exposure to fruit.
- Canopy management practices, such as shoot tucking, can help minimize the direct sun exposure to fruits.
- Sunblock sprays, such as Kaolin and CaCO3, increase reflectance and thereby reduce solar heating of fruit and leaves.
- Evaporative cooling, such as in-canopy micromisting, can be effective, but water use and disease pressure could be increased.
- Last but not least, adequate irrigation to develop the canopy which can help shade the fruit and protect fruits.
Among all the above options, irrigation might be the most important to prevent fruit sunburn during heat waves, since the sufficient canopy promoted by irrigation does not only serve as the photosynthetic machinery to produce carbohydrate to ripen fruits, but also provides the shade for fruits to reduce excessive sun exposure. There are many irrigation tools which growers can use to watch out for potential water deficit in their vineyards:
- Soil moisture-based irrigation.
- Plant water-based irrigation.
- Weather-based irrigation.
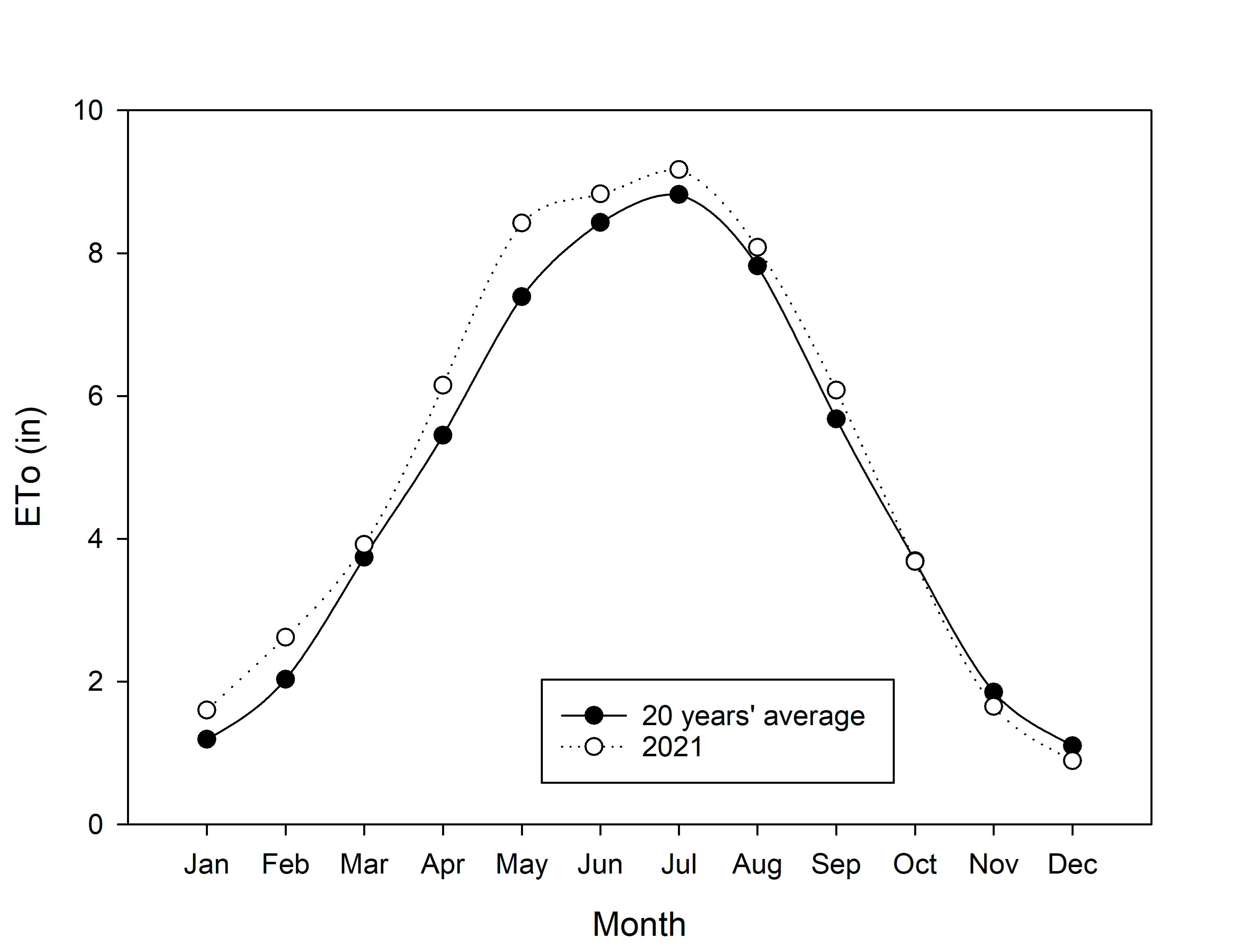
Most growers I have talked to have soil moisture sensors on-site, and currently, CDFA offers various grants (cdfa.ca.gov/oefi/sweep/) to help growers install soil moisture sensors.
No matter which soil moisture sensor you have, the key is to identify the soil moisture benchmark which you can target the irrigation to. So, how can soil moisture sensors help you to target the benchmark and manage the water in your vineyard? For example, based on Figure 1 (see page 26), from May 2021 to August 2021, growers utilized the soil moisture sensor (soil water volumetric sensor, Campbell Scientific CS655) to schedule the irrigation to maintain the soil moisture content range from 10% to 15% at soil depth of two feet.
On this site, three soil water volumetric sensors were installed at approximately one foot from the vine trunk and six inches from the emitter. Sensors were set at the depth of one, two and three feet beneath the soil surface. We assume growers are satisfied with the canopy and crop development during the season, and a field study done by Dr. Larry Williams at UC Kearney REC also shows 10% to 15% soil moisture content correlates to -1.2 to -0.9 MPa midday leaf water potential (Williams 2012). The range from -1.2 to -0.9 MPa of midday leaf water potential is regarded as mild water stress or no water stress for the SJV vines and can be used to maximize the crop production (Figure 4).
Given the soil type on this site is similar to the Hanford fine sandy loam soil in UC Kearney REC, the same range of soil moisture content can serve as a good benchmark for any future irrigation scheduling on this site. Please note that no water applied after mid-August aimed to prepare the soil for raisin drying. Unsurprisingly, in 2022, growers started the early spring irrigation in mid-February to target the same range of soil moisture content from 10% to 15% to prepare the budbreak and early shoot development. Therefore, growers can irrigate the grapevines by selecting the desired soil moisture benchmark based on the preferred canopy and crop development on your specific soil type.
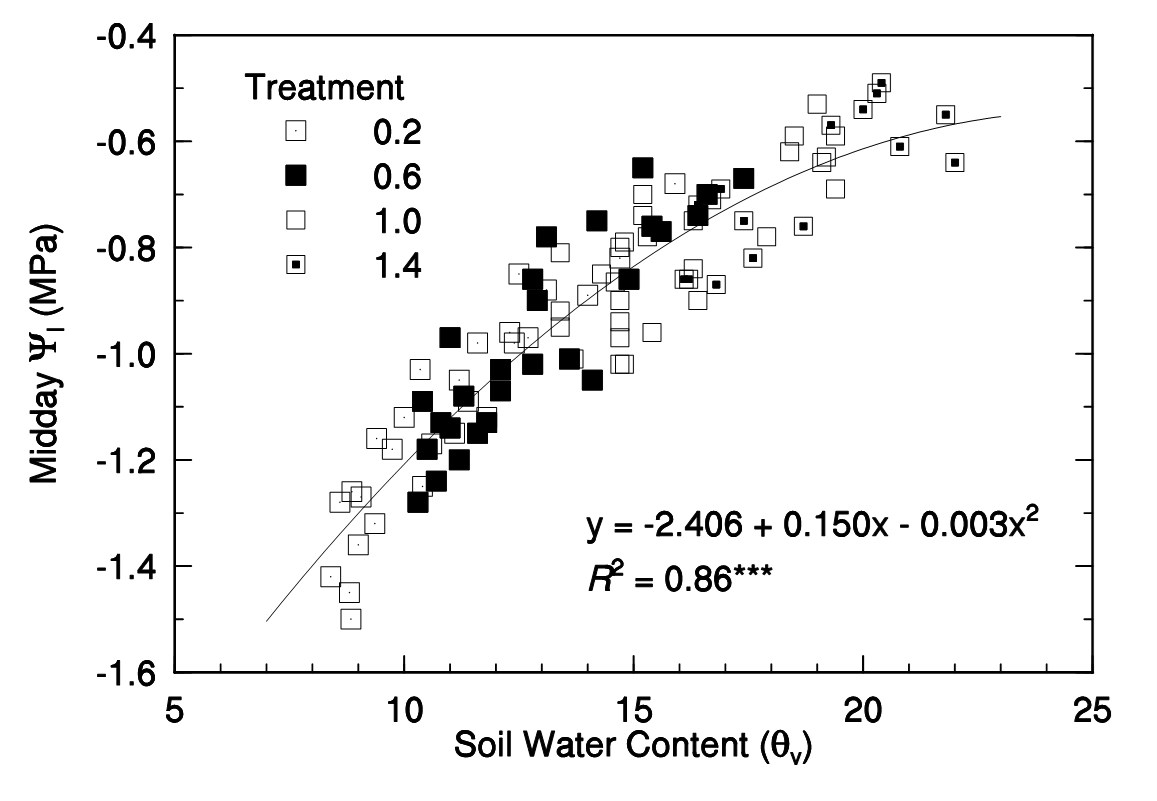
Nutrient Management During Drought
Low rainfall in autumn to midwinter can cause drought-induced boron deficiency. The symptoms are erratic budbreak, stunted and distorted shoots, misshapen and chlorotic leaves. The most classic symptoms after budbreak are dwarfed shoots that grow in a zigzag manner with numerous lateral shoots, and the tip of the primary shoot may die. Most shoots begin to elongate normally by late spring, but cluster size may be reduced. The cause is believed to be a late-season drought-induced boron deficiency that affects development of shoots within dormant buds.
The key to reduce drought-induced boron deficiency is postharvest irrigation. Traditionally, Thompson raisin vineyards go through the harvest and raisin-drying processes for a nearly two-month period without irrigation (Figure 1, see page 26). The postharvest irrigation can relieve the water stress and maintain a healthy functional canopy to avoid boron deficiency.
Spring fever is sometimes referred to as false potassium deficiency because the leaf symptoms resemble and are sometimes confused with potassium deficiency. Alternating warm and cold weather patterns before bloom, as has been observed this spring, can cause a temporary nitrogen metabolism disorder associated with high levels of ammonium and the polyamine putrescine in the leaves.
Symptoms occur in basal leaves and leaves in the fruit zone. Lower leaf color fades and becomes chlorotic in spring, beginning at the leaf margins and progressing between the primary and secondary veins. Leaf margins may become slightly necrotic, marginal necrosis is significant and affected leaves can drop.
There is no cure for spring fever, and petiole/blade laboratory analysis can differentiate true K deficiency from spring fever. Spring fever typically will fade as the weather warms up and the onset of symptomatic leaves decreases around bloom; however, blades with existing symptoms will remain.
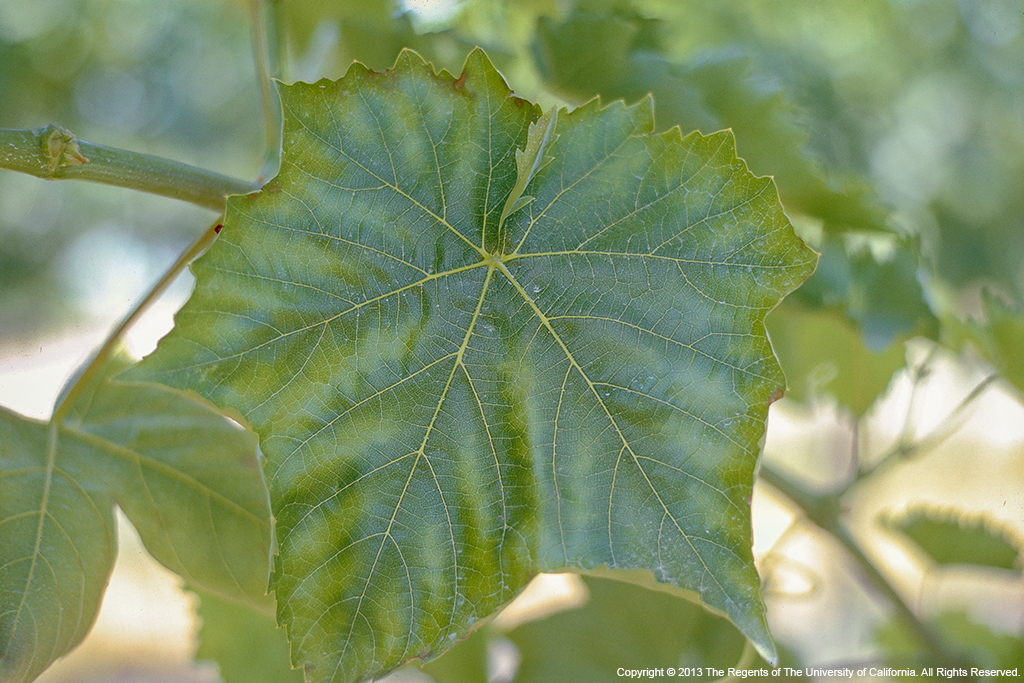
References
Bettiga, Larry. Grape Pest Management, Third Edition. University of California Agriculture and Natural Resources. 2013.
Christensen, Pete. Raisin Production Manual. University of California Agriculture and Natural Resources. 2000.
Williams, L. (2012), Effects of applied water amounts at various fractions of evapotranspiration (ETc) on leaf gas exchange of Thompson Seedless grapevines. Australian Journal of Grape and Wine Research, 18: 100-108. https://doi.org/10.1111/j.1755-0238.2011.00176.x